Cathodic Polarization and Bacteria-accelerated Corrosion of X65 Steel
Introduction
Cathodic polarization is an effective and common method to prevent corrosion of metallic structures and marine facilities.[1] The protection potential of −770 mV/SCE at room temperature can resist the naturally occurring corrosion current and prevent the steel from corrosion risk.[2]
However, there have been some unaccountably external corrosion problems, and in many cases, the presence of microorganisms (e.g., sulfatereducing bacteria [SRB]) is associated with these problems.[3] Microbiologically induced corrosion (MIC) is a bioelectrochemical process that initiates or accelerates the corrosion reaction by microbial activity.[4,5] MIC induced by SRB has been extensively investigated in the literature. Zhu et al.[8] found that the corrosion rate of X56 steel in the sea mud containing SRB was 10‐fold higher than in the sea mud without SRB. SRB can reduce sulfate (SO42−) to sulfide (H2S, HS−), which may indirectly contribute to the accumulation of corrosive sulfide and organic acid end products, causing localized pitting of metals.[9] Several mechanisms have been proposed to explain the role of SRB in MIC,[10-15] including cathodic depolarization, local corrosive cell, and metabolite‐induced corrosion. Cathodic depolarization accelerates the cathodic reaction due to the consumption of cathode hydrogen via hydrogenase biocatalysis by SRB, which increases the electron demand of the anode and associated corrosion.[16,17] Metal sulfides produced by SRB metabolism are electrically conductive and cathodic with respect to steel, forming aggressive corrosion cells.[14] Recently, it has been suggested that SRB can acquire the energy needed by obtaining electrons via direct or indirect contact with the steel substrate, leading to more severe corrosion.[18]
Studies have shown that the electrochemical changes at the metal–solution interface during cathodic polarization influence the composition and concentration of chemical species and the attachment of microorganisms.[19-21]. Sun et al.[22] studied the effects of SRB on cathodic polarization of Q235 steel in the soils and showed that the number of SRB in soils decreased, and the protection efficiency increased with the shift of applied potential to a negative direction. Saravia et al.[23] stated that there was a decrease in the number of attached cells when the cathodic potential was applied in the initial stages of biofilm formation. Olivares et al.[24] , however, presented opposite findings. They found that the SRB population on polarization potential‐applied pipeline steel (−850mV vs. Cu/CuSO4) was twice that of without cathodic polarization. Liu et al.[25] confirmed that the application of cathodic polarization did not affect the growth of planktonic bacteria, but it was beneficial to the adhesion of SRB cells to steel in the extracted soil solution.
To date, the interaction between cathodic polarization and microbial activity has not been well clarified due to the complicated processes occurring at the cathodically polarized surface. The objective of this study was to investigate the effect of cathodic polarization on microbial attachment and the effect of various cathodic potentials on the corrosion of X65 steel in sea water containing SRB.
Materials and Methods
2.1 Materials
Fluorine‐doped tin oxide (FTO) conducting glass (10 × 20 mm) was used for fluorescent confocal imaging to study the effect of cathodic current on SRB cells. Another specimen was X65 pipeline steel, which was used to study the effect of cathodic potential on corrosion in the presence of SRB. The X65 specimen (10 × 10 × 3 mm) was machined and embedded in epoxy resin, leaving a work area of 1 cm2 for electrochemical measurement. The work face of the specimen was mechanically abraded with silicon carbide metallurgical papers sequentially, degreased by anhydrous ethanol, and then dried in high-purity nitrogen. All the prepared specimens were sanitized for 30 min using an ultraviolet lamp before use to ensure that there was no contamination by other bacteria.
2.2 SRB strain and its inoculation process
In this study, SRB (i.e., Desulfovibrio caledoniensis) were isolated from the rust layers of carbon steel immersed in seawater (Qingdao, China) and identified by polymerase chain reaction (PCR) amplification of 16S ribosomal DNA. SRB strains were cultivated in a constant‐temperature incubator at 30 °C (86 °F) for 5 days. Then, 5 ml of SRB strains were transferred into 500 ml seawater (containing the whole culture medium) to prepare the test solution. Before inoculation, the medium was deoxygenated with high‐purity nitrogen for 30 min and then sterilized by autoclaving at 121 °C (249.8 °F) for 20 min. The living cell number of SRB was calculated by the most probable number ASTM standard D4412‐84.
2.3 Surface analysis
Bacterial attachment on the FTO glass electrode surface in situ was characterized using a confocal laser scanning microscope (FLUOVIEW™ FV1000; Olympus®). Before observation, the electrodes were soaked in sterile phosphate‐buffered saline solution containing 5% (v/v) glutaraldehyde for 30 min and then stained with a fluorescent dye (DAPI) for 30 min in darkness.
The surface morphologies of X65 steel were observed using a scanning electron microscope (SEM; JSM‐6700F; JEOL). The elemental composition and valence of the corrosion products were measured by energy‐dispersive X‐ray spectroscopy (EDS; JSM‐6700F; JEOL) and X‐ray photoelectron spectroscopy (XPS; ESCALAB 250Xi; Thermo Fisher Scientific).
A three‐dimensional measuring laser microscope (LEXT™ OLS4000; Olympus, Tokyo, Japan) was used to characterize the surface morphology of the corroded steel specimens after the removal of corrosion products. Before the corroded surface examination, the specimens were taken from the test solution and subsequently immersed in a pickling solution containing corrosion inhibitor (hexamethylenetetramine) for 5 min, then rinsed with distilled water, cleaned with absolute ethanol, and finally dried with a nitrogen gas stream.
2.4 Electrochemical measurements
All the electrochemical measurements were performed using a Gamry electrochemical workstation. A saturated calomel electrode and a platinum plate were used as the reference and counter electrode, respectively. X65 steel was cathodically polarized for 14 days at the potentials of −800 and −1,050 mV/SCE, respectively. In addition, the cathodic potentials of −1,050 mV/SCE (the first 7 days) to −800 mV/SCE (the last 7 days) and −800 mV/SCE (the first 7 days) to −1,050 mV/SCE (the last 7 days) were also applied. Electrochemical impedance spectroscopy (EIS) was performed when the open circuit potential (OCP) reached a steady state. The applied polarization potential was switched off for a certain period to allow the potential to return to OCP, and then the EIS measurement was performed. After the EIS test was completed, the applied polarization potential was again switched on. EIS was obtained in the frequency range of 105–10−2 Hz, and the amplitude of the sinusoidal voltage signal was 10 mV. The EIS data were analyzed using Zview2 software with a suitable equivalent circuit model. All electrochemical experiments were carried out at 25 °C (77 °F) in an airtight system and repeated at least three times to check the reproducibility.
Results and Discussion
3.1 The growth curve of SRB
The growth curve of SRB displayed a typical three‐stage growth cycle. The first stage, which lasted for 1–4 days, is the exponential growth phase. During this stage, the number of active SRB increased rapidly. The growth stage from 5–10 days is the stationary phase. During this stage, the number of SRB increased and decreased equally, so the total number of SRB was large and stable. After 10 days, the growth process reached the death phase, during which the number of SRB decreased dramatically due to the lack of essential nutriments.
3.2 The effect of cathodic current on bacterial attachment
After applying different cathodic current for 30 hr, the effect of electric quantity on the attachment of SRB to the FTO electrode was studied, as given in Figure 1. The results showed that when more negative charges were applied, less SRB cells were attached. When the electric quantity of 400 mA/m2 ×30 hr) was applied, the attachment of SRB on the electrode surface was significantly less than that of the control system (no current). The electric quantities of 300 mA/m2×30 hr and 200 mA/m2×30 hr had a little influence on the adhesion degree of SRB. Wilson et al.[26] have reported that cell surface was negatively charged by ionized phosphoryl and carboxylate substituents under normal conditions. Therefore, SRB cells may be repelled by a large build‐up of negative charges on the surface of cathodically polarized electrode.[27] When the electric quantity applied to the FTO increased, there was an increase in the electrostatic repulsion. Consequently, the attachment of SRB was inhibited by the large electric quantity (400 mA/m2×30 hr). Interestingly, when a relatively small electric quantity was applied to the surface of the FTO electrode (Figure 1d), the number of attached SRB was more than that of the control system.
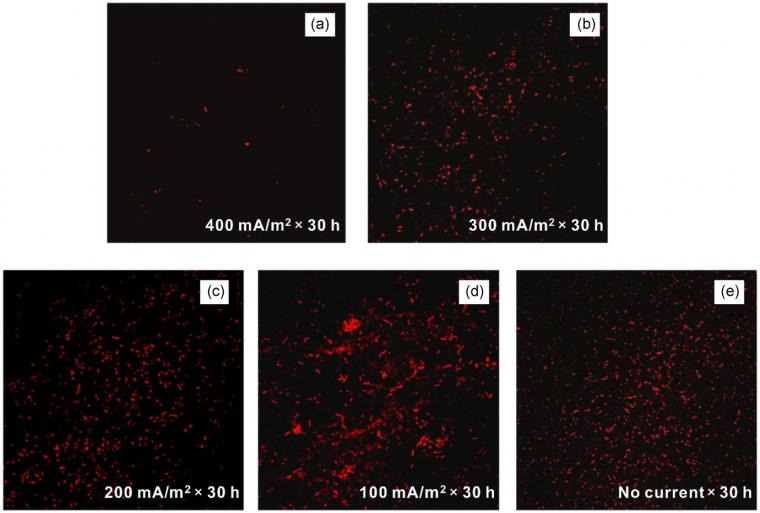
These results indicated that electric quantity was an important factor affecting the attachment of SRB. However, when the same electric quantity was applied, was microbial attachment related to current intensity? Figure 2 shows the attachment of SRB on the surface of FTO electrode at the same electric quantity, but at various current densities. As shown in Figure 2a–d, the number of attached SRB cells markedly decreased with the increase of current density. The high cathodic current of 400 mA/m2 effectively inhibited bacterial attachment and subsequent biofilm formation. It was further demonstrated that although the same quantity of electric charge was applied, a low current of 100 mA/m2 promoted bacterial attachment. When the electrostatic force between FTO and SRB was insufficient, cathodic polarization promoted the evolution of hydrogen, which contributed to the growth of hydrogenase‐positive SRB.[28] Thus, there was an increase in SRB cells attached to the FTO at 100 mA/m2. Given the above analysis, the attachment of SRB can be properly controlled by adjusting the electric quantity in combination with a high current.
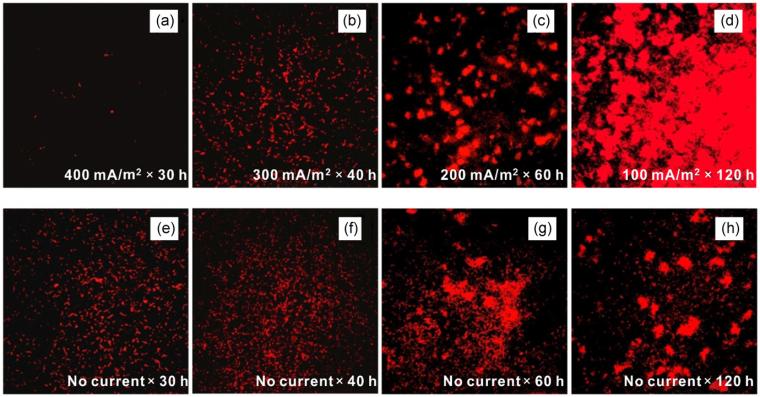
3.3 The effect of cathodic potential on the corrosion of X65 steel in SRB‐containing seawater
Most microorganisms tend to colonize, proliferate, and form a biofilm on metal surfaces, resulting in MIC. The initial attachment of bacteria is a crucial step, which lays the foundation for the formation of biofilm. Therefore, it is important to prevent or delay bacterial attachment and growth for corrosion control. In marine engineering, the commonly used criterion for the effectiveness of cathodic protection is the polarization potential. Therefore, −800 and −1,050 mV/SCE were selected as the applied potentials for this study. In addition, the cathodic potential ranging from −1,050 to −800 mV/SCE was impressed to X65 steel, that is, the negative potential of −1,050 mV/SCE was used to inhibit bacterial attachment in the rapid growth phase of SRB, and the inhibition effect of −800 mV/SCE on corrosion in the later stage was studied. The cathodic potential shifted negatively from −800 to −1,050 mV/SCE.
3.3.1 OCP monitoring
The different OCP variations in both systems, SRB‐inoculated medium and sterile medium, indicated that the activity of SRB affected the corrosion process of X65 steel. The OCP of X65 steel in the sterile system remained stable at around −670 mV/SCE. In contrast, the OCP values in the SRB system fluctuated significantly and moved negatively to −718 mV/SCE after 5 days. It was possible that the activity of SRB changed the electrochemical process, providing a high driving force for the corrosion of X65 steel. Subsequently, the OCP moved to a positive direction and stabilized at the potential of around −640 mV/SCE.
3.3.2 EIS measurements
For specimens in the SRB‐containing medium at OCP, the diameter of the Nyquist plot increased from 1 to 4 days, then decreased with time gradually, and afterward increased again after 14 days. The increase of the Nyquist plot diameter was attributed to the formed biofilm and corrosion products providing some protection to the steel in the initial incubation time, which hindered the charge transfer process. The decrease of the Nyquist plot diameter indicated that the high SRB activity accelerated the corrosion of X65 steel. Meanwhile, the production of corrosive agents such as sulfide, organic acids, and/or inorganic acids by SRB promoted the anode dissolution of metal.[7,15] After 14 days, the Nyquist plot diameter increased again due to the decline in the SRB activity. The peak of the phase angle shifted to a high frequency before 4 days and then to a low frequency. This situation indicated the formation of a protective corrosion product film, followed by the breakdown of the film. In the presence of SRB at cathodic potential of −800 mV/SCE, the diameter of the Nyquist plot was several times smaller than that at OCP, indicating that this cathodic potential had no protective effect on SRB‐induced corrosion. When the potential of −1,050 mV/SCE was applied on the electrode, the diameter of Nyquist plot increased gradually. The impedance values were larger than that at OCP, which indicated that this potential effectively slowed down the corrosion in the presence of SRB. The potential ranging from −1,050 to −800 mV/SCE also offered some protection to the specimen toward further corrosion. The Nyquist plot for −800 to −1,050 mV/SCE was relatively volatile, and there may be a slight anodic dissolution.
The time‐dependent changes of Rct fitted from the EIS data at various cathodic potentials was studied. Generally, a higher Rct indicates a lower corrosion rate of steel. At OCP, the Rct increased and reached the maximum value after 4 days, and then decreased gradually. The initial increase of Rct can be attributed to the production of biofilm and corrosion products that formed a protective film on the specimen surface. After 4 days, the number of active SRB increased rapidly and reached the maximum value at 7 days, which accelerated the corrosion of X65 steel. In addition, the integrity of the protective film may be degraded, thereby forming an active corrosion cell between the FeS film (cathode) and the nearby metal substrate (anode), which resulted in a rapid decrease of Rct. However, in the case of the cathodic polarization, Rct was increased when the polarization potential shifted negatively. The results were well matched with the cathodic protection theory mentioned in Evan's diagram; that is, the increase of protective potential increased the corrosion resistance. The Rct with the highest increase was observed at −1,050 mV/SCE. It revealed that anodic dissolution was almost inhibited at the cathodic potential of −1,050 mV/SCE.
3.3.3 Current densities' recording
It was observed that the current density decreased rapidly within a few hours and reached a steady state. Additionally, the cathodic current increased after a few days. This increase was suggested to result from the following factors: (a) cathodically produced hydrogen promoted an SRB activity and (b) iron sulfides produced by SRB were cathodic, relative to X65 steel, which may form a corrosion cell, resulting in a higher cathodic current.[29]
3.3.4 pH variation
The pH values decreased abruptly in the SRB‐containing culture solution with bacterial growth and then increased. The initial drop in pH values can be attributed to the generation of organic acids. SRB consumed cathodic hydrogen through a hydrogen intermediate (i.e., hydrogenase) for the reduction of SO42−, as given below:
(1) SO42− + 8H+ + 8e− → HS− + 3H2O + OH−
The reaction increased the concentration of OH− because it consumed H+, increasing, the pH of the solution. Although the changes in pH for all systems exhibited a similar pattern, the pH values at various polarization potentials were higher than that at OCP. This indicated that the alkalinity generated by cathodic polarization was higher than that by microbiological activity.
3.3.5 Surface analysis
Figure 3 shows the SEM images of X65 specimens at various polarization potentials after 14 days of exposure in SRB‐containing seawater. A large number of loosely discrete products were attached to the surface at OCP (Figure 3a). Numerous SRB cells were embedded in the corrosion products and biofilm matrix. The EDS results showed that the elements of corrosion products were mostly Fe, C, O, and S. Thus, the corrosion products were principally iron oxides, sulfides, as well as carbon‐based compounds that accumulated from the culture medium. When the cathodic potential of −800 mV/SCE was applied on the electrode, the corrosion product layer was more compact (Figure 3b). It is observed that SRB cells still existed among the corrosion products. At −1,050 mV/SCE, dense deposits were formed on the X65 steel surface (Figure 3c) and no bacteria were observed on the surface. The EDS analysis indicated the presence of calcareous deposits and a low amount of iron sulfides. The formation of calcareous deposits on the electrode surface was the result of negative protection potential producing high pH value, which was beneficial to the deposition of calcium ions. At −1,050 to −800 mV/SCE, there were obvious corrosion cracks on the surface, which were caused by drying during specimen preparation (Figure 3d). At −800 to −1,050mV/SCE, the steel electrode contained a layer of corrosion products (Figure 3e) with extensive SRB cells.
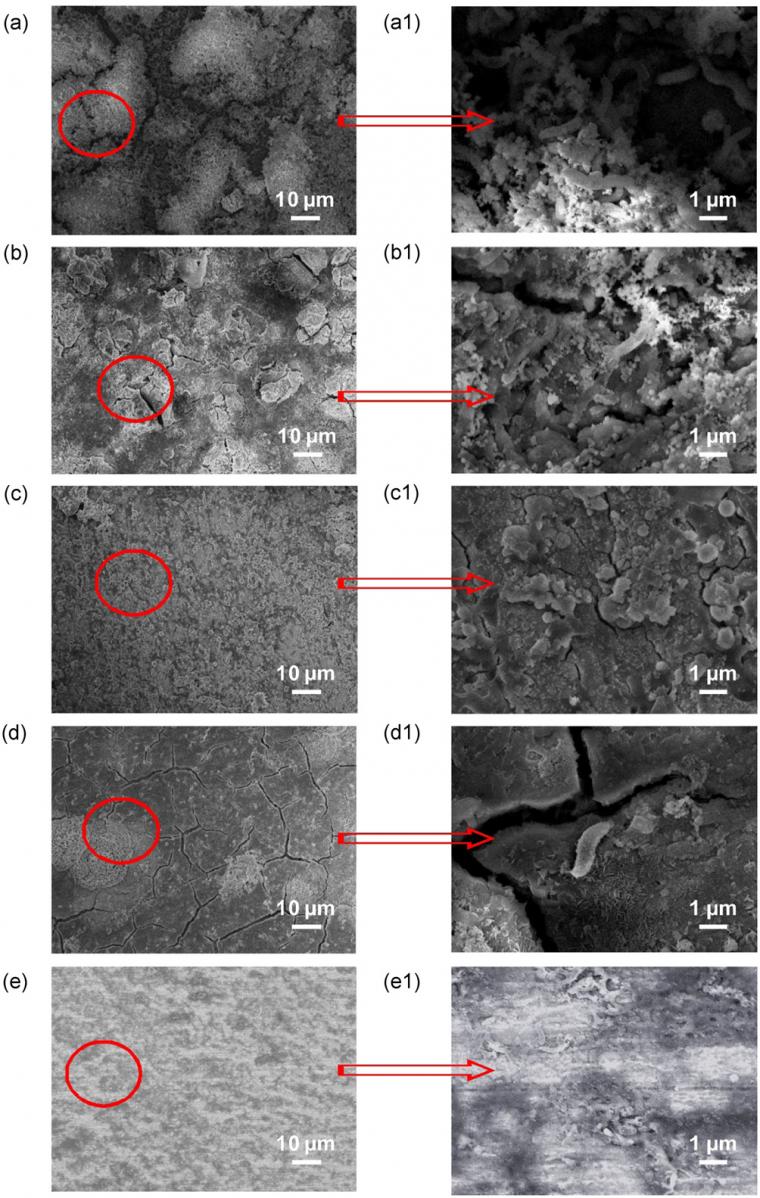
Corrosion morphologies of X65 steel after removing corrosion products are shown in Figure 4. Some big and deep pits were observed at OCP (Figure 10a), which was attributed to anodic dissolution occurring on the steel surface and SRB‐induced pitting. At cathodic potential of −800 mV/SCE (Figure 10b), pitting corrosion still occurred. By contrast, the surface morphology of the specimen at −1,050 mV/SCE was, in general, smooth with no apparent pitting corrosion (Figure 10c). It was consistent with the electrochemical result that corrosion was prevented by a cathodic potential of −1,050 mV/SCE. At −1,050 to −800 mV/SCE (Figure 4d), the general corrosion of X65 steel was effectively controlled, except for a few small pits. When the cathodic potential shifted negatively from −800 to −1,050 mV/SCE, these pits seemed to be wider but not deeper than that at −800 mV/SCE (Figure 4e).
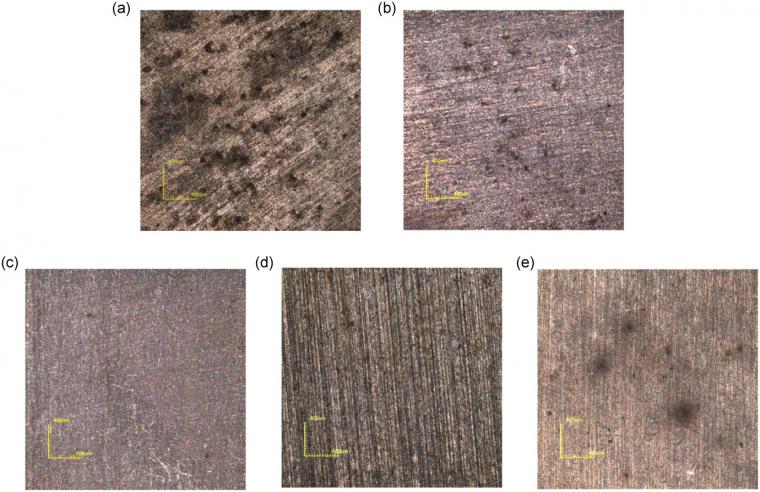
3.3.6 XPS analysis
XPS spectra were employed to qualitatively and quantitatively determinate the corrosion products of the X65 specimen. For the Fe spectrum of corrosion products, FeSO4, Fe2O3, FeOOH, FeS, and FeS2 were detected in almost all specimens. Interestingly, some iron sulfides were also found at the cathodic potential of −1,050 mV/SCE. It can be hypothesized that a negative potential of −1,050 mV/SCE can play a better protective effect, but it cannot completely eliminate the occurrence of MIC.
3.3.7 The influence of cathodic potential on SRB‐induced corrosion
The SEM micrographs in this study showed a significant reduction of the bacterial number on the cathodic surface polarized to −1,050 mV/SCE. For most cathodic surfaces in the anaerobic condition, an impressed cathodic potential forced the following reactions:
(2) 2H2O + 2e− → H+ + 2OH−.
In this case, the production of OH− caused an increase of interfacial pH, which seemed high enough to inhibit the initial attachment of SRB. Furthermore, the surface charge on bacteria cells depended on interfacial pH, which determined the degree of protonation of ionogenic groups associated with the cell wall to be negatively charged. The microbial cell produced a negatively charged polysaccharide, glycocalyx; thus, its attachment to the polarized surface was electrostatically repelled when a cathodic potential of −1,050 mV/SCE was applied.[30,31]
Calcareous deposits were formed on the surface of the cathodically polarized electrode. The increase in local pH, in turn, forced a shift in the equilibria of all chemical reactions involving calcium, magnesium, and carbonate:
(3) HCO− + OH− → H2O + CO32−
(4) Mg2+ + 2OH− → Mg(OH)
(5) Ca2+ + CO32− → CaCO3
Cations such as Ca2+ and Mg2+ are required for bacterial adhesion as a “bridge.”[32] The precipitation of Ca and Mg ions caused a decrease in their local concentration due to the generation of OH−, thereby inhibiting the attachment of SRB to the surface of cathodically polarized X65 steel. These calcareous deposits also provided a physical barrier to general corrosion attack. At the cathodic potential of −1,050 mV/SCE, the formation of calcareous deposits and the alkaline environment on the surface of the polarized electrode was not suitable for SRB to obtain electrons from the overly negative polarized X65 steel and grow. According to the corrosion morphology of X65 steel after removing corrosion products (Figure 4c), the protection potential of −1,050 mV/SCE was sufficient to control the corrosion induced by SRB.
The bacterial activity was not affected by the protection potential of −800 mV/SCE applied to the X65 steel. Besides the general corrosion observed on the metal surface, a type of localized corrosion was developed when the potential of −800 mV/SCE was applied to the SRB system (Figure 4b). Thus, −800 mV/SCE was not sufficient to inhibit SRB‐induced corrosion. Some works have found that cathodic hydrogen generation at the cathodically protected surface promoted the growth of hydrogenase-containing SRB.[32] Chemical species (including H+ ions) were reduced at the metal surface:
(6) H+ + e− → [H].
The generated atoms [H] were transported to the biofilm and then oxidized by hydrogenase enzyme to reduce SO42−. Further, H+ protons were adsorbed on the metal surface and reduced again. It was apparent that the cathodic potential changed the bacterial metabolism and consequently affected the current demand.
However, several studies indicated that SRB can also obtain electrons from metallic iron directly via membrane-associated proteins (e.g., cytochromes) to reduce SO42−.[33-35] Therefore, the cathodic polarization potential must be lower than the optimal protection criterion for steel due to the presence of SRB.
In the experiment conducted at the cathodic potential ranging from −800 to −1,050 mV/SCE, the SEM observation (Figure 3e) indicated that SRB strongly attached to the electrode surface, despite the fact that potential negatively shifted to −1,050 mV/SCE after 7 days. The reason was suggested that cathodic polarization was not sufficient to remove bacteria from the pre‐existing biofilm.[29] When a potential of −1,050 to −800 mV/SCE was applied, the protective effect was similar to that at −1,050 mV/ SCE. It indicated that the changes in the metal interface caused by −1,050 mV/SCE were sufficient to suppress SRB‐induced corrosion for a period of time after the potential shifted to −800 mV/SCE. However, long‐term predictions require further research in SRB‐containing seawater.
Conclusions
In this study, it was demonstrated that the degree of SRB attachment on the electrode surface was associated with the electric quantity and the intensity of the cathodic current. Due to the build‐up of negative charges on the electrode surface, the cathodic current of 400mA/m2×30 hr effectively inhibited bacterial attachment. In contrast, when a low cathodic current of 100mA/m2 was applied, bacterial attachment and growth were promoted. Also, the attachment of SRB on the electrode surface was not inhibited by the potential of −800mV/SCE. The generated atoms [H] on the polarized surface contributed to the metabolic activity of SRB. A further negative shift of the protection potential to −1,050mV/SCE exhibited an effective inhibitory effect on SRB‐induced pitting corrosion. The impressed charges, the increase of pH, and the formation of calcareous deposits on the electrode surface were the driving forces to inhibit the attachment of SRB. The cathodic potential ranging from −1,050 to −800mV/SCE also provided effective protection for X65 steel due to the interfacial changes caused by potential of −1,050mV/SCE in the first 7 days. However, at the potential ranging from −800 to −1,050mV/SCE, pitting corrosion still existed on the electrode surface, indicating that the applied potential of −1,050mV/SCE could inhibit SRB attachment, but it was not sufficient to remove SRB from the pre‐existing biofilm.
References
[1] Y. S. Kim, S. K. Lee, H. J. Chung, J. G. Kim, Ocean Eng. 2018, 148, 223.
[2] J. H. Kim, Y. S. Kim, J. G. Kim, Ocean Eng. 2016, 115, 149.
[3] R. G. Esquivel, G. Z. Olivares, M. J. H. Gayosso, A. G. Trejo, Mater. Corros. 2011, 62, 61.
[4] C. H. Williamson, L. A. Jain, B. Mishra, D. L. Olson, J. R. Spear, Appl. Microbiol. Biotechnol. 2015, 99, 6945.
[5] R. Javaherdashti, Appl. Microbiol. Biotechnol. 2011, 91, 1507.
[6] K. M. Moon, H. R. Cho, M. H. Lee, S. K. Shin, S. C. Koh, Met. Mater. Int. 2007, 13, 211.
[7] F. M. AlAbbas, R. Bhola, J. R. Spear, D. L. Olson, B. Mishra, Int. J. Electrochem. Sci. 2013, 8, 859.
[8] Y. Zhu, Y. Huang, C. Zheng, Q. Yu, Mater. Corros. 2007, 58, 447.
[9] S. Y. Li, K. S. Jeon, T. Y. Kang, Y. T. Kho, Y. G. Kim, Corrosion 2001, 57, 815.
[10] V. W. Kuehr, V. D. Vlugt, Water (den Haag) 1934, 18, 147.
[11] S. Daumas, M. Magot, J. L. Crolet, Res. Microbiol. 1993, 144, 327.
[12] D. Thierry, W. Sand Corrosion Mechanisms in Theory and Practice (Eds: P. Marcus, J. Oudar), Marcel Dekker, New York, NY 1995.
[13] D. Thierry, W. Sand Corrosion Mechanisms in Theory and Practice, 2nd ed. (Ed: P. Marcus), Marcel Dekker, New York, NY 2002.
[14] W. A. Hamilton, Biofouling 2003, 19, 65.
[15] R. Jia, J. L. Tan, P. Jin, D. J. Blackwood, D. Xu, T. Gu, Corros. Sci. 2018, 130, 1.
[16] T. Q. Wu, M. C. Yan, D. C. Zeng, J. Xu, C. K. Yu, C. Sun, W. Ke, Acta Metall. Sin. 2014, 28, 93.
[17] H. A. Videla, Biofouling 2009, 15, 37.
[18] P. Zhang, D. Xu, Y. Li, K. Yang, T. Gu, Bioelectrochemistry 2015, 101, 14.
[19] S. H. Hong, J. Jeong, S. Shim, H. Kang, S. Kwon, K. H. Ahn, J. Yoon, Biotechnol. Bioeng. 2008, 100, 379.
[20] O. Istanbullu, J. Babauta, H. Duc Nguyen, H. Beyenal, Biofouling 2012, 28, 769.
[21] S. Shim, S. H. Hong, Y. Tak, J. Yoon, Biofouling 2011, 27, 217.
[22] C. Sun, J. Xu, F. H. Wang, C. K. Yu, Mater. Corros. 2010, 61, 762.
[23] S. G. G. de Saravia, M. F. L. de Mele, H. A. Videla, R. G. J. Edyvean, Biofouling 1997, 11, 1.
[24] G. Olivares, G. Mejia, G. Caloca, I. Lopez, F. Dabur, C. Ulloa‐Ochoa, R. Esquivel, Corrosion 2003.
[25] T. Liu, Y. F. Cheng, J. Alloy. Compd. 2017, 729, 180.
[26] W. W. Wilson, M. M. Wade, S. C. Holman, F. R. Champlin, J. Microbiol. Methods 2001, 43, 153.
[27] A. T. Poortinga, J. Smit, H. C. V. D. Mei, H. J. Busscher, Biotechnol. Bioeng. 2001, 76, 395.
[28] F. Guan, X. Zhai, J. Duan, M. Zhang, B. Hou, PLOS One 2016, 11, e0162315.
[29] J. G. Guezennec, Int. Biodeterior. Biodegrad. 1994, 34, 275.
[30] B. Little, P. Wagner, D. Duquette, Corrosion 1988, 44, 270.
[31] R. G. J. Edyvean, A. D. Maines, C. J. Hutchinson, N. J. Silk, L. V. Evans, Int. Biodeterior. Biodegrad. 1992, 29, 251.
[32] J. Guezennec, Biofouling 1991, 3, 339.
[33] J. Duan, S. Wu, X. Zhang, G. Huang, M. Du, B. Hou, Electrochim. Acta 2008, 54, 22.
[34] H. Venzlaff, D. Enning, J. Srinivasan, K. J. J. Mayrhofer, A. W. Hassel, F. Widdel, M. Stratmann, Corros. Sci. 2013, 66, 88.
[35] H. T. Dinh, J. Kuever, M. Mußmann, A. W. Hassel, M. Stratmann, F. Widdel, Nature 2004, 427, 829.
Original article:
Lv, M, Li, X, Du, M. The effect of cathodic polarization on the corrosion behavior of X65 steel in seawater containing sulfate-reducing bacteria. Materials and Corrosion. 2020; 71: 2038– 2051. https://doi.org/10.1002/maco.202011715
Source: Preview Image: Shannon Stent/iStockphoto